伽马氧化铝(γ-Al2O3)结构和功能关系
Zhenchao Zhao, Dong Xiao, Kuizhi Chen, Rui Wang, Lixin Liang, Zhengmao Liu, Ivan Hung, Zhehong Gan, and Guangjin Hou
Five-coordinated Als (Al(V)) on the surface of aluminas play important roles when they are used as catalysts or catalyst supports. However, the comprehensive characterization and understanding of the intrinsic structural properties of the Al(V) remain a challenge, due to the very small amount in commonly used aluminas. Herein, the surface structures of γ-Al2O3 and Al(V)-rich Al2O3 nanosheets (Al2O3–NS) have been investigated and compared in detail by multinuclear high-field solid-state NMR. Thanks to the high resolution and sensitivity of ultra-high-field (up to 35.2 T) NMR, the arrangements of surface Als were clearly demonstrated, which are substantially different from the bulk phase in γ-Al2O3 due to the structure reconstruction. It reveals for the first time that most of the commonly observed Al(V)s tend to exist as aggregated states on the surface of γ-Al2O3, like those in amorphous Al2O3–NS liable to structure reconstruction. Our new insights into surface Al(V) species may help in understanding the structure–function relationship of alumina.
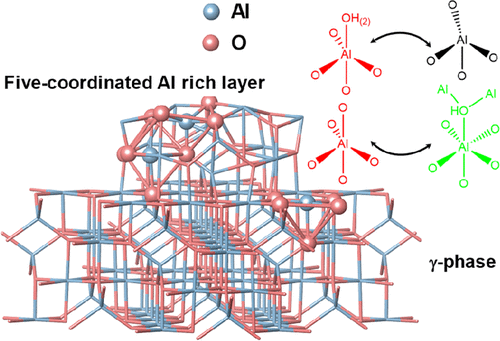
Introduction
Transition aluminas with different structures and morphologies have been widely used as catalytic materials. As a typical alumina with high surface area and thermal stability, γ-Al2O3 has shown extensive applications in hydrodesulfurization, syngas to methanol, DeNOx, and propane dehydrogenation reactions as industrial catalyst supports. (1,2) The intrinsic Lewis acid sites (three-, four-, and five-coordinated Al) in γ-Al2O3 confer them with good acid catalytic properties for alcohol dehydration, olefin isomerization reactions, etc. (1) Because of its important practical applications, extensive studies on the structure of surface Al and hydroxyl species have been carried out utilizing infrared spectroscopy (IR), solid-state NMR spectroscopy, high-resolution transmission electron microscopy (TEM), temperature-programmed desorption, as well as theoretical calculations, to get a deeper understanding of the active sites for catalytic reactions and anchor sites when they are used as catalysts or catalyst supports. (3−10) Nevertheless, the intrinsic low crystallinity makes detailed structural characterization of Al on the surface of γ-Al2O3 at the atomic level extremely challenging. (11−13) Five-coordinated Al (Al(V)), claimed as “Super 5”, is a very important surface Al species for both the active site for dehydration reactions and the anchor site for loading catalysts. (4) For example, the surface Al(V) Lewis acid sites are ascribed to the active sites for alcohol dehydration reaction. (14,15) It is also suggested that Al(V)s on alumina are the binding sites of active metal atoms such as Pt, Pd, Ru, etc. (16−20) Indeed, various Al2O3 with abundant Al(V) were synthesized and proved to be good catalysts or catalyst supports. (21−24) Besides, Al(V) on the surface of γ-Al2O3 was also found to be very critical for phase transformation from γ-Al2O3 to α-Al2O3, which is strongly related to its thermal stability as catalyst support. (21,25) Theoretical calculations demonstrate that Al(V)s exist on both the (100) surface and microfacets (111) in the reconstructed surface of γ-Al2O3. (26−29) Very recently, it was proposed that the macroscopically defined (110) surfaces in rhombus-platelet γ-Al2O3 can be reconstructed into (111) and (100) segments, where Al(V) was derived from six-coordinated Al (Al(VI)) at the (100) surface by removal of hydroxyl groups. (30) However, the intrinsic structure related to Al(V) of γ-Al2O3 is far from well-known, as it is a very small amount in γ-Al2O3, while almost all the reported Al(V)-rich aluminas are in the amorphous phase.
Solid-state NMR spectroscopy is a very powerful tool for exploring the local structure of materials at the atomic level regardless of their crystalline or amorphous properties. (31−33) Various 27Al NMR techniques including 27Al MAS, 1H–27Al CP/MAS, 1H–27Al HMQC, 27Al DQ, and MQ MAS NMR have been used to study alumina materials. (4,34) For commonly used γ-Al2O3, Al(V) presents only on the surface and in a very small amount, and its contribution to the signals in the one-pulse 27Al MAS NMR spectra is negligible and difficult to study in detail. (35) Recently, it was reported that 1H–27Al HMQC or CP/MAS NMR can significantly enhance the signals of Al(V), and two kinds of Al(V) species with significantly different quadrupolar coupling constants (Qcc) (10 MHz Vs 1–3 MHz) were proposed. (36−38) However, suffering from low intensity and spectral broadening for 27Al NMR, very few detailed studies on the intrinsic structures related to these Al(V) species have been reported. With the advance of ultra-high-field NMR technology, the detection sensitivity and spectral resolution of the quadrupolar nucleus can be significantly enhanced, providing an opportunity for detailed characterization of Al(V).
Herein, we carried out a comparative investigation on Al(V)-rich Al2O3–NS and γ-Al2O3 thermally treated under conditions similar to their practical applications, to elucidate the structural characteristics of Al(V) in γ-Al2O3. Benefiting from the significantly enhanced sensitivity and resolution of the ultra-high field up to 35.2 T, 27Al–27Al DQ/SQ correlation NMR experiments allowed us to explore direct Al–O–Al connections on the surface of γ-Al2O3. By using advanced multinuclear and multidimensional MAS NMR techniques, surprisingly similar features regarding the coordination environment of surface Als and their corresponding hydroxyl groups, selective removal of hydroxyl groups, as well as the distribution of Al(V), were unraveled for γ-Al2O3 and Al2O3–NS.
Results and Discussion
TEM images and XRD patterns of γ-Al2O3 and Al2O3–NS are shown in Figure S1 and S2, respectively. γ-Al2O3 shows irregular aggregates of nanocrystals, and Al2O3–NS shows a long thin sheet morphology as the amorphous phase similar to previous reports. (39)27Al MAS NMR spectra of γ-Al2O3 and Al2O3–NS before and after dehydration at different temperatures and the relative amount of each Al species analyzed by dmfit software according to 27Al MQ MAS NMR (Figure S3) are shown in Figure 1. (40) The pristine γ-Al2O3 (Figure 1a) shows two main peaks at 11 and 69 ppm and minor signals at 35 ppm, corresponding to six(VI)-, four(IV)-, and five(V)-coordinated Al, respectively. After dehydration at 350 and 550 °C, there is only a slight decrease of Al(VI) accompanied by the increase of Al(IV). As only a very small amount of Al(V) is present in γ-Al2O3, the quantitative analysis of this Al species is challenging, and opposite trends were reported during the dehydration or preparation of catalysts. (16,41,42) In contrast, for pristine Al2O3–NS (Figure 1b), there is significant signal intensity for the Al(V) at 37 ppm, and the signal of Al(VI) appears at a relatively higher field near 7 ppm. After dehydration at 350 °C, the relative amount of Al(VI) decreases significantly and Al(V) becomes dominant (about 50%). More specifically, the Al(VI) decreases by about 36%, Al(V) and Al(IV) species increase by about 23% and 13%, respectively. The results clearly demonstrate that Al(V) may be formed from the hydration of Al(IV) or dehydration of Al(VI). Although a very small change of the amount of Al(V) for γ-Al2O3 was seen, its signal was broadened significantly upon the dehydration treatment (Figures 1a and S3a–c). It suggests that Al(V) is different for γ-Al2O3 under different dehydration status, implying some Al(V) was transformed into Al(IV) and also formed from Al(VI) simultaneously.
Figure 1
Figure 1. 27Al MAS NMR spectra and the corresponding deconvolutions of pristine (bottom) and dehydrated aluminas at 350 °C (middle) and 550 °C (top), γ-Al2O3 (a) and Al2O3–NS (b), acquired at 18.8 T and MAS rate of 20 kHz. Note that the absolute errors for the deconvolution are ±0.2% for Al(V) and ±1% for Al(V, VI) in γ-Al2O3, while for Al2O3–NS, the absolute errors are about ±3% due to the spectral overlap.
1H MAS NMR spectra of pristine and dehydrated Al2O3 samples are shown in Figure 2, where the intensity or vertical scale is normalized to the sample weight. The pristine γ-Al2O3 and Al2O3–NS show signals of hydrogen-bonded and physisorbed water at 4.5 and 4.8 ppm, respectively. (41) After dehydration at 350 °C, for both γ-Al2O3 and Al2O3–NS, three main groups of signals are observed at −0.2 to 0.3, 0.8 to 2.0, and above 2.5 ppm, corresponding to isolated terminal, double-, and triple-bridged hydroxyl groups, respectively. (36) It is noted that the major signals of hydroxyl groups in Al2O3–NS near 0.3 and 2.0 ppm are also present in γ-Al2O3. However, the relative intensities are different, and specifically, the major signal of the terminal hydroxyl locates at 0.3 ppm for Al2O3–NS but −0.2 ppm for γ-Al2O3, and the main double-bridged hydroxyl groups present at 2.0 ppm for Al2O3–NS but 1.6 ppm for γ-Al2O3. When elevating the dehydration temperature to 550 °C, most hydroxyl groups (∼70% according to integrated area compared with 350 °C dehydrated sample) were desorbed for Al2O3–NS. On the contrary, only the signals at 0.3, 1.6, and ∼3.0 ppm hydroxyl groups on γ-Al2O3 decrease apparently. The results clearly indicate that the surface hydroxyl groups in γ-Al2O3 have significantly different thermal stability, although the overall stability is much better than those of amorphous Al2O3–NS.
Figure 2
Figure 2. 1H MAS NMR spectra of pristine (bottom) and dehydrated aluminas at 350 °C (middle) and 550 °C (top), γ-Al2O3 (a) and Al2O3–NS (b), recorded at 18.8 T and MAS rate of 20 kHz.
In order to probe the spatial distribution of different hydroxyl groups on the alumina surface, 1H–1H DQ/SQ correlation NMR experiments were further carried out, and the spectra are shown in Figure 3. The correlation peaks mean that the spin pairs have close spatial proximity. It is worth noting that the terminal hydroxyl groups (−0.2 to 0.3 ppm) in γ-Al2O3 show very weak autocorrelation signals (i.e., signals arising from chemically equivalent species in close proximity) along with the diagonal, and a very weak correlation peak (2.0 ppm, 0.3 ppm) was also observed. Three strong correlation peaks between terminal hydroxyl and double-bridged hydroxyl groups (A: −0.2 to 0.3 ppm, 1.6 ppm), double-bridged and double-bridged hydroxyls (B: 1.2 ppm, 1.6 ppm), as well as triple-bridged hydroxyl groups and double-bridged hydroxyl (C: 2.5–5 ppm, 1.6 ppm) are observed. The results demonstrate that the double-bridged hydroxyl at 1.6 ppm correlated with all the other hydroxyl groups in γ-Al2O3. As for Al2O3–NS, strong autocorrelation peaks were observed for terminal (D: 0.3 ppm, 0.3 ppm) and double-bridged hydroxyl groups (E: 1.2 ppm, 1.2 ppm). In addition, the spatial proximities between the terminal hydroxyl and bridged hydroxyl groups (F: 2.0 ppm, 0.3 ppm) and double-bridged hydroxyl groups (G: 1.6 ppm, 2.0 ppm) were observed. It is worth noting that both samples show strong correlations for the signals near 1.2–2.0 ppm (B and G), which means that these double-bridged hydroxyl groups have close spatial proximity to each other. The proximity of hydroxyl groups may accommodate the dehydration process at high temperatures. For instance, the hydroxyl groups at 1.2–1.6 ppm are mainly hydroxyl groups removed after 550 °C dehydration for γ-Al2O3, as shown in Figure 2a. This is also the case for Al2O3–NS, where most hydroxyl groups were removed after 550 °C dehydration, due to the spatial proximity for all of them.
Figure 3
Figure 3. 1H–1H DQ/SQ correlation NMR spectra of 350 °C dehydrated γ-Al2O3 (a) and Al2O3–NS (b) acquired at 18.8 T and MAS rate of 20 kHz. The BABA sequence was used for 1H–1H dipolar recoupling, and the mixing time was 0.4 ms.
The information on connectivity between hydroxyl groups and surface Al species can be obtained by performing 2D 1H–27Al correlation NMR spectroscopy, where the through-space D-RINEPT (refocused insensitive nuclei enhanced by polarization transfer) (43,44) method was used. Both 27Al and 1H detected 1H–27Al D-RINEPT NMR spectra were acquired, as shown in Figure 4. 27Al detected 1H–27Al D-RINEPT spectrum of dehydrated γ-Al2O3 (Figure 4a) indicates that the terminal hydroxyl groups at −0.2 ppm correlate with Al(IV), and the double-bridged hydroxyl groups at 1.6 ppm correlate with Al(V) and Al(VI), while the double-bridged hydroxyl groups near 2.0 ppm correlate with Al(IV). The triple-bridged hydroxyl groups above 2.5 ppm are mainly correlated with Al(VI). These results are consistent with previous reports. (36) Thanks to the higher sensitivity and resolution of 1H dimension in the 1H detected D-RINEPT spectrum (Figure 4b), additional correlations can be unambiguously discriminated between terminal hydroxyl groups at 0.3 ppm and Al(IV). Moreover, the correlation peak between triple-bridged hydroxyl groups above 2.5 ppm and Al(V) was also observed. In comparison, on the surface of Al2O3–NS (Figure 4c), the terminal hydroxyl groups at 0.3 ppm correlate with both Al(IV) and Al(V), while double-bridged hydroxyl groups at 2.0 ppm correlate with all Al(IV), Al(V), and Al(VI), whereas the 1H detected D-RINEPT spectrum (Figure 4d) shows that the double-bridged hydroxyl groups at 1.6 ppm mainly correlate with Al(VI) and the triple-bridged hydroxyl groups above 2.5 ppm mainly correlate with Al(V). It is worth noting that all the hydroxyl–aluminum correlations in Al2O3–NS are observable in γ-Al2O3 (clearly shown in 1H detected D-RINEPT spectra Figure 4b and d), and the only difference is that they have different relative intensities, whereas 1H–27Al D-RINEPT spectra of both pristine samples (Figure S4) indicate that surface Al(IV) and Al(V) show similar features with signifcantly narrower 27Al peaks due to smaller QCC.
Figure 4
Figure 4. 1H–27Al D-RINEPT correlation NMR spectra of 350 °C dehydrated γ-Al2O3 (a,b) and Al2O3–NS (c,d) using 27Al (left) and 1H (right) detection acquired at 18.8 T and MAS rate of 20 kHz. The dipolar recoupling SR412 sequence was applied during the mixing periods, and the optimized recoupling times were 0.6 and 1.0 ms for 27Al and 1H detected D-RINEPT NMR experiments, respectively.
In order to obtain the information on the connectivity of Al with different coordination environments for pristine and dehydrated Al2O3, 27Al–27Al DQ/SQ NMR experiments were further carried out. It should be noted that 27Al–27Al DQ/SQ NMR signals are very sensitive to Al–Al distance (see Figure S5 for details). As shown in 27Al–27Al DQ/SQ NMR spectra of γ-Al2O3 with different RF carrier frequencies (Figure S6a–c), although the DQ excitation efficiency is also affected by the carrier frequency, it can still be concluded that Al(VI) was spatially correlated with Al(IV), indicative of the off-diagonal peaks, and also strongly autocorrelated, indicative of the diagonal peak. In contrast to the previous report, the weak correlation among Al(IV) was also observed when the center of RF was moved near Al(IV). (45) The absence of all correlation peaks related to Al(V) may be due to its very small amount in γ-Al2O3 and large quadrupolar interaction. In comparison, 27Al–27Al DQ/SQ NMR spectrum of Al2O3–NS (Figure S6d–f) demonstrates that each aluminum species has autocorrelation, and the correlations with the other two Al species, similar to previously reported Al(V)-rich amorphous mesoporous alumina utilizing the dynamic nuclear polarization (DNP) technique. (46) It means that all the connectivities among Al(VI), Al(V), and Al(IV) exist for amorphous mesoporous alumina and Al2O3–NS, but the question whether Al(V) on the surface of γ-Al2O3 has similar features as Al2O3–NS remains to be answered. In order to have efficient NMR sensitivity and resolution, we further carried out 27Al MAS and DQ/SQ NMR experiments for dehydrated γ-Al2O3 and Al2O3–NS at the currently accessible highest field (35.2 T) spectrometer, and the corresponding spectra are shown in Figure 5. As shown in 1D 27Al MAS NMR spectra (Figure 5a,b), the significantly enhanced spectral resolution was achieved at the ultrahigh magnetic field. Note that the five-coordinated Al takes 3.5% (±0.2%) of total Als γ-Al2O3, which is slightly different from that derived at 18.8T (Figure 1), and the similar slight deviation was observed for Al2O3–NS. It should be expected that the significantly improved spectral resolution at ultra-high field can lead to higher accuracy on quantitative analysis. For the 27Al–27Al DQ/SQ spectrum (Figure 5c), autocorrelations of Al(IV) and Al(VI) and correlation between Al(IV) and Al(VI) from bulk phase were observed to be similar to that of pristine γ-Al2O3. Surprisingly, Al(V) in γ-Al2O3 presents a strong autocorrelation peak comparable to much more abundant Al(IV) and Al(VI), even though it only accounts for 3.5% of the total Al (Figure 5a), which was never observed even using the DNP NMR technique. (35) In addition, the correlation peak between Al(V) and Al(IV) is also observed. Note that with the RF carrier frequency closer to Al(VI), the correlation peaks with Al(VI) become stronger, and the weak correlation between Al(V) and Al(VI) can also be observed (Figure S7). For dehydrated Al2O3–NS, the 27Al–27Al DQ/SQ NMR experiment (Figure 5d) demonstrates that Al(V) and Al(IV) have strong autocorrelation signals, as well as a strong correlation between them. However, weak autocorrelation peaks were barely observed for Al(VI) due to the resonance offset. Compared with the 27Al 1D MAS NMR spectra of γ-Al2O3 and Al2O3–NS, there is a significant enhancement of 27Al DQ MAS NMR signals of Al(V) for γ-Al2O3 but not for Al2O3–NS, although the RF carrier frequencies for both samples are the same. Therefore, the RF carrier frequency does not play a dominant role at this point. Since Al(V) were proposed to only exist on the surface of γ-Al2O3, the presence of these correlation peaks of surface Al species (Al(V)–Al(V), Al(V)–Al(IV), Al(V)–Al(VI)) highly suggests that these spin pairs have closer spatial proximity than those in the bulk phase. It is in accordance with previous reports that the enhanced contrast of surface in TEM images was due to the modified surface structures with excess cations (more dense Al3+) at the surface of γ-Al2O3. (47) These 27Al–27Al DQ/SQ NMR results clearly illustrate that Al(V) in γ-Al2O3 have the same characteristics as Al2O3–NS with respect to Al connectivities (highlighted by red in Figure 5); that is, Al(V)s are spatially autocorrelated and also correlated with Al(IV).
Figure 5
Figure 5. Ultra-high-field 2D 27Al–27Al DQ/SQ correlation (bottom) and corresponding 1D 27Al MAS NMR spectra (top) of 350 °C dehydrated γ-Al2O3 (a,c) and Al2O3–NS (b,d) acquired at 35.2 T and MAS rate of 30 kHz. The BR221 sequence with RF field strength of 15 kHz was used for 27Al–27Al dipolar recoupling, and the recoupling time was 0.8 ms.
1H–1H DQ/SQ and 1H–27Al D-RINEPT NMR demonstrate that the connectivities between Al and hydroxyl of γ-Al2O3 contain all the features that are present on Al2O3–NS. 27Al–27Al DQ/SQ NMR results suggest that the Al–O–Al connectivities found in Al2O3–NS (highlighted by red in Figure 5) are also included in γ-Al2O3 excluding bulk Al(VI)–Al(VI) and Al(IV)–Al(VI) connectivities. All these results allow us to infer that the surface structures related to Al(V) in γ-Al2O3 should be similar to those in Al2O3–NS. For both γ-Al2O3 and Al2O3–NS, various Al(V)s should exist: (1) Al(V) from Al(IV) due to adsorption of water; (2) Al(V) from Al(VI) after dehydration by removing bridged hydroxyls. Possible models for the formation of Al(V)s are demonstrated in Scheme 1a–e, while only Al(V)s with smaller quadrupolar interaction are observed in 27Al MQ MAS NMR (Figure S3). Two kinds of Al(V)s were proposed from 1H–27Al HMQC/CP MAS NMR, i.e., one with strong quadrupolar interaction (10 MHz); the other with weak quadrupolar interaction (1–3 MHz) for dehydrated γ-Al2O3. (36,38) Theoretically, Al(V)s with square pyramidal structure are mainly present on the surface of (100), where only Al(V)s were exposed. (28,30) Al(V)–O–Al(V) (derived from Al(VI) by dehydration with the shortest Al–Al distance, Scheme 1a) connectivities at the surface of (100) can well explain the strong autocorrelation of five-coordinated Al in 27Al–27Al DQ/SQ NMR. If Al(V)s with square pyramidal structure were mainly derived from the dehydration of surface Al(VI)s with terminal hydroxyl groups after dehydration above 375 °C as previously reported, (30) the correlation peaks between Al(VI) and terminal hydroxyl groups should be clearly observed in 1H–27Al D-RINEPT NMR at moderate dehydration temperature of 350 °C. On the contrary, the 1H–27Al D-RINEPT NMR results indicate that the terminal hydroxyl groups are mainly correlated with Al(IV) and Al(V) (Figure 4). In fact, Al(V) species with high symmetry were proposed to exist and even observed in liquid solutions, and they are also stable on the surface of hydrated alumina. (48−50) Al(V) in γ-Al2O3 exposed at ambient conditions might be mainly from the Al(IV) after adsorption of water (Scheme 1b), which shows symmetric signals in 27Al MAS NMR (Figure 1 and Figure S4). The surface reconstruction via the removal of bridged hydroxyl groups of Al(VI)s also forms Al(V)s (Scheme 1c,d) with strong quadrupolar interactions (Figure 1 and Figure 4) on γ-Al2O3, which should be another reasonable explanation for the formation of the Al(V) dominant structure upon the dehydration, as revealed by 1H MAS NMR and predicted by theoretical calculations. (51) As is clearly shown in 27Al MAS NMR, the dehydration treatment leads to the structural reconstruction in Al2O3–NS, where about 77% Al(VI) was transformed into Al(IV)/Al(V). Once most of the hydroxyl groups were removed, no significant changes of Al coordination status were observed upon further dehydration even at 550 °C. Moreover, when Al2O3–NS was wetted by water, almost all the Al(IV)s and Al(V)s could be transformed into Al(VI) (Figure S8). The flexibility of Al coordination for Al(V)-rich alumina was also previously reported for ρ-alumina, which was too sensitive to moisture to be transformed into hydroxides and oxyhydroxides with Al(VI). (4,52) It was further confirmed by 1H–27Al D-RINEPT spectra that the surface Al experience significantly different quadrupolar interactions for both samples in pristine and dehydrated status (Figures 4 and S4). Therefore, the structures of most Al(V) at the surface of γ-Al2O3 are highly similar to the Al(V) dominant structures such as amorphous Al2O3–NS. Indeed, very high Al(V) content was reported on the deposited amorphous Al2O3 thin films as well as mechanically milled γ-Al2O3. (21,53,54) In fact, we can roughly estimate that the relative amount of Al(V) on the surface of γ-Al2O3 can reach up to 26% via ultra-high-field 1H–27Al HMQC NMR spectra (Figure S9), which is an appreciable amount of the total surface Al. Our findings on the extraordinary coordination environment, Al(V) related connections, and viable structure reconstruction may confer distinct roles on Al(V) for active sites, binding sites, or as nucleation center for sintering of alumina. (15,16,25)
Scheme 1
Scheme 1. Proposed Models for the Formation of Five-Coordinated Al
Conclusions
The structural properties of Al(V) species in alumina were comprehensively studied by a combination of high-field multinuclear and multidimensional MAS NMR. The results demonstrate that amorphous Al2O3–NS can be a representative phase to the commonly observed surface Al(V) in γ-Al2O3. Quantitative 27Al MAS NMR indicates the flexible coordination numbers of Al changing from six, five, to four for both aluminas. 1H MAS and DQ/SQ NMR data demonstrate the hydroxyl groups on the surface of γ-Al2O3 with close spatial proximity that are viable to be removed under high-temperature dehydration resulting in surface structure reconstruction. Moreover, it is evidenced for the first time that most Al(V) species tend to aggregate into Al(V) domains on the surface of γ-Al2O3 like Al2O3–NS, rather than tetragonal pyramid coordination on the (100) surface previously predicted from theoretical models. It is believed that these new insights into surface Al(V) species would help to understand the structure–function relationship of Al2O3 when used as catalyst and catalyst supports.
Supporting Information
The Supporting Information is available free of charge at https://pubs.acs.org/doi/10.1021/acscentsci.1c01497.
XRD patterns, TEM images, 27Al MAS and MQ MAS NMR spectra, 1D 1H–27Al HMQC MAS NMR, 27Al–27Al DQ MAS NMR spectra (PDF)
Nature of Five-Coordinated Al in γ‑Al2O3 Revealed by Ultra-High-Field Solid-State NMR
S1 Supplementary Information for:OntheNature of Five-coordinate Alin g-Al2O3Revealed by Ultra-high Field Solid-state NMRZhenchao Zhao1, Dong Xiao1, Kuizhi Chen1,2, Rui Wang1, Lixin Liang1, ZhengmaoLiu1, Ivan Hung2, Zhehong Gan2, Guangjin Hou1*1. State Key Laboratory of Catalysis, Dalian Institute of Chemical Physics, Chinese Academy of Sciences, 457 Zhongshan Road, Dalian 116023, China2. National High Magnetic Field Laboratory, 1800 East Paul Dirac Drive, Tallahassee, FL 32310, United StatesExperimentalNo unexpected or unusually high safety hazards were encountered during all the experimental procedures.g-Al2O3 (Alfa Asear) was first calcined at 550 °C under static air before NMR characterization. Five-coordinate Al rich Al2O3-NS was synthesized according to previous report.1Typically, 6.5 g Al(NO3)3×9H2O (AR, Sinopharm Chemical Reagent Co., Ltd) and 9.2g urea (AR, Sinopharm Chemical ReagentCo., Ltd) were dissolved into 60 ml deionized water under magnetic stirring until a clear solution was obtained. The solution was then transferred into a 100 ml autoclave and kept in a 100 °C oven for 48 h. Then the precipitates were obtained by repeated washing with deionized water and filtration, and dried at 110 °Cfor 12 h. Finally, it was calcined at 600 °C for 2 h with a ramp rate of 2 °C/min. TEM images were obtained on a HitachHT7700 Transmission Electron Microscope at accelerating voltage of 100 kV. Powder X-Ray diffraction (XRD) patterns were collected on the Rigaku D/ MAX-2500 X-ray diffractometer with Cu Kα radiation in the 2θrange of 10−70° and a scan rate of 5°/min.The solid samples were dehydrated under high vacuum of 10-4Pa at 350 or 550 °C for 16 and 5 h, respectively, with a temperature ramp rate of 2 °C/min. 350 °C is the typical working temperatures when aluminas are used as alcohol dehydration catalysts, and 550 °C is the representative calcination temperature when they are used as catalyst supports.
S2 the dehydrated samples were packed into NMR rotors in an Ar glove-box avoiding exposure of air and moisture. Solid-stateNMR experiments were carried out on three NMR spectrometers. For 27Al and 1H MAS NMR spectra were acquired at a Bruker AVANCE NEO800 spectrometerwith the resonance frequency of 208.5 and 800.3 MHz, respectively. A3.2 mmHXdouble resonance probe was used at a MASrate of 20 kHz. 1H MAS NMR spectra were acquired using csecho pulse sequence for background suppression with a 1H 90°pulse of 3.3μs, and 32scans were accumulated with a 2-10s recycle delay. 27Al MAS NMR spectra were acquired using a small flip angle of π/18 pulse of 0.5μs, and 256scans were accumulated with a 2s recycle delay. 1H-27AlDR-INEPTNMRwere set up with a 27Al selective central transition π/2 pulse of 16 μs. The dipolar recoupling SR421 sequence was applied with recoupling time of 0.6 to 1.4 ms. 192 to 768scans were accumulated for each t1 increment, and a recycle delay of 2 to 10 s was used.Recoupling times were set around 1 and 0.6 ms for27Aland1H detected D-RINEPT NMR experiments, respectively.1H-1H DQ MAS NMR were acquired using BABApulse sequence, 64scans were accumulated for each t1 increment. 27Al DQ MASNMRspectra of g-Al2O3and as-prepared Al2O3-NS wasacquired using BR2!”recouplings2with a 27Al selective central transition π/2 pulse of 10 μs, 1536 scans were accumulated for each t1 increment, and a recycle delay of 1.2 s was used.27Al MQMAS NMR spectra were acquired at a Bruker AVANCE IIIHD600 spectrometerat the resonance frequency of 156.4MHz using a 3.2 mmHXYtriple resonance probe at a MASrate of 21.4kHz. A 3-pulse sequence (P1 = 3.6 μs,P2 = 5.8μs,P3= 21 μs) with double frequency sweep (DFS) enhancement (mp3qdfsz) was used for 2D data acquisition.3, 4480 scans were accumulated for each increment with a 0.5 s recycle delayand64 evolution increments were used. Ultra-high field (35.2T) solid-state 27Al MAS,1H-27Al HMQC and 27Al DQMAS NMR spectra were acquired using the series-connected hybrid (SCH) magnet at the National High Magnetic Field Laboratory (NHMFL) in Tallahassee, FL, USA. A Bruker Avance NEO
Terms & Conditions
Most electronic Supporting Information files are available without a subscription to ACS Web Editions. Such files may be downloaded by article for research use (if there is a public use license linked to the relevant article, that license may permit other uses). Permission may be obtained from ACS for other uses through requests via the RightsLink permission system: http://pubs.acs.org/page/copyright/permissions.html.
Author Information
Corresponding Author
Guangjin Hou – State Key Laboratory of Catalysis, Dalian Institute of Chemical Physics, Chinese Academy of Sciences, 457 Zhongshan Road, Dalian 116023, China; Orcidhttps://orcid.org/0000-0001-8216-863X; Email: ghou@dicp.ac.cn
Authors
Zhenchao Zhao – State Key Laboratory of Catalysis, Dalian Institute of Chemical Physics, Chinese Academy of Sciences, 457 Zhongshan Road, Dalian 116023, China
Dong Xiao – State Key Laboratory of Catalysis, Dalian Institute of Chemical Physics, Chinese Academy of Sciences, 457 Zhongshan Road, Dalian 116023, China; Orcidhttps://orcid.org/0000-0003-1485-4915
Kuizhi Chen – State Key Laboratory of Catalysis, Dalian Institute of Chemical Physics, Chinese Academy of Sciences, 457 Zhongshan Road, Dalian 116023, China; National High Magnetic Field Laboratory, 1800 East Paul Dirac Drive, Tallahassee, Florida 32310, United States; Orcidhttps://orcid.org/0000-0002-9853-7070
Rui Wang – State Key Laboratory of Catalysis, Dalian Institute of Chemical Physics, Chinese Academy of Sciences, 457 Zhongshan Road, Dalian 116023, China
Lixin Liang – State Key Laboratory of Catalysis, Dalian Institute of Chemical Physics, Chinese Academy of Sciences, 457 Zhongshan Road, Dalian 116023, China
Zhengmao Liu – State Key Laboratory of Catalysis, Dalian Institute of Chemical Physics, Chinese Academy of Sciences, 457 Zhongshan Road, Dalian 116023, China; Orcidhttps://orcid.org/0000-0002-4597-0022
Ivan Hung – National High Magnetic Field Laboratory, 1800 East Paul Dirac Drive, Tallahassee, Florida 32310, United States; Orcidhttps://orcid.org/0000-0001-8916-739X
Zhehong Gan – National High Magnetic Field Laboratory, 1800 East Paul Dirac Drive, Tallahassee, Florida 32310, United States; Orcidhttps://orcid.org/0000-0002-9855-5113
Notes
The authors declare no competing financial interest.
References
This article references 54 other publications.
1Pines, H.; Haag, W. O. Alumina: Catalyst and Support. I. Alumina, Its Intrinsic Acidity and Catalytic Activity. J. Am. Chem. Soc. 1960, 82, 2471– 2483, DOI: 10.1021/ja01495a021 [ACS Full Text ACS Full Text], [CAS], Google Scholar
2Trueba, M.; Trasatti, S. P. γ-Alumina as a Support for Catalysts: A Review of Fundamental Aspects. Eur. J. Inorg. Chem. 2005, 2005, 3393– 3403, DOI: 10.1002/ejic.200500348 [Crossref], Google Scholar
3Digne, M.; Sautet, P.; Raybaud, P.; Euzen, P.; Toulhoat, H. Hydroxyl Groups on γ-Alumina Surfaces: A DFT Study. J. Catal. 2002, 211, 1– 5, DOI: 10.1006/jcat.2002.3741 [Crossref], [CAS], Google Scholar
4Chandran, C. V.; Kirschhock, C. E. A.; Radhakrishnan, S.; Taulelle, F.; Martens, J. A.; Breynaert, E. Alumina: Discriminative Analysis Using 3D Correlation of Solid-State NMR Parameters. Chem. Soc. Rev. 2019, 48, 134– 156, DOI: 10.1039/C8CS00321A [Crossref], [PubMed], [CAS], Google Scholar
5Boumaza, A.; Favaro, L.; Lédion, J.; Sattonnay, G.; Brubach, J. B.; Berthet, P.; Huntz, A. M.; Roy, P.; Tétot, R. Transition Alumina Phases Induced by Heat Treatment of Boehmite: An X-Ray Diffraction and Infrared Spectroscopy Study. J. Solid State Chem. 2009, 182, 1171– 1176, DOI: 10.1016/j.jssc.2009.02.006 [Crossref], [CAS], Google Scholar
6Joubert, J.; Fleurat-Lessard, P.; Delbecq, F.; Sautet, P. Simulating Temperature Programmed Desorption of Water on Hydrated γ-Alumina from First-Principles Calculations. J. Phys. Chem. B 2006, 110, 7392– 7395, DOI: 10.1021/jp056633n [ACS Full Text ACS Full Text], [CAS], Google Scholar
7Amenomiya, Y.; Cvetanović, R. J. Active Sites of Alumina and Silica-Alumina as Observed by Temperature Programmed Desorption. J. Catal. 1970, 18, 329– 337, DOI: 10.1016/0021-9517(70)90327-1 [Crossref], [CAS], Google Scholar
8Kovarik, L.; Genc, A.; Wang, C.; Qiu, A.; Peden, C. H. F.; Szanyi, J.; Kwak, J. H. Tomography and High-Resolution Electron Microscopy Study of Surfaces and Porosity in a Plate-Like γ-Al2O3. J. Phys. Chem. C 2013, 117, 179– 186, DOI: 10.1021/jp306800h [ACS Full Text ACS Full Text], [CAS], Google Scholar
9Xu, S.; Jaegers, N. R.; Hu, W.; Kwak, J. H.; Bao, X.; Sun, J.; Wang, Y.; Hu, J. Z. High-Field One-Dimensional and Two-Dimensional 27Al Magic-Angle Spinning Nuclear Magnetic Resonance Study of Θ-, Δ-, and γ-Al2O3 Dominated Aluminum Oxides: Toward Understanding the Al Sites in γ-Al2O3. ACS Omega 2021, 6, 4090– 4099, DOI: 10.1021/acsomega.0c06163 [ACS Full Text ACS Full Text], [CAS], Google Scholar
10Wang, Q.; Li, W.; Hung, I.; Mentink-Vigier, F.; Wang, X.; Qi, G.; Wang, X.; Gan, Z.; Xu, J.; Deng, F. Mapping the Oxygen Structure of γ-Al2O3 by High-Field Solid-State NMR Spectroscopy. Nat. Commun. 2020, 11, 3620, DOI: 10.1038/s41467-020-17470-4 [Crossref], [PubMed], [CAS], Google Scholar
11Paglia, G.; Božin, E. S.; Billinge, S. J. L. Fine-Scale Nanostructure in γ-Al2O3. Chem. Mater. 2006, 18, 3242– 3248, DOI: 10.1021/cm060277j [ACS Full Text ACS Full Text], [CAS], Google Scholar
12Samain, L.; Jaworski, A.; Edén, M.; Ladd, D. M.; Seo, D.-K.; Javier Garcia-Garcia, F.; Häussermann, U. Structural Analysis of Highly Porous γ-Al2O3. J. Solid State Chem. 2014, 217, 1– 8, DOI: 10.1016/j.jssc.2014.05.004 [Crossref], [CAS], Google Scholar
13Prins, R. Location of the Spinel Vacancies in γ-Al2O3. Angew. Chem., Int. Ed. 2019, 58, 15548– 15552, DOI: 10.1002/anie.201901497 [Crossref], [CAS], Google Scholar
14Larmier, K.; Chizallet, C.; Cadran, N.; Maury, S.; Abboud, J.; Lamic-Humblot, A.-F.; Marceau, E.; Lauron-Pernot, H. Mechanistic Investigation of Isopropanol Conversion on Alumina Catalysts: Location of Active Sites for Alkene/Ether Production. ACS Catal. 2015, 5, 4423– 4437, DOI: 10.1021/acscatal.5b00723 [ACS Full Text ACS Full Text], [CAS], Google Scholar
15Hu, J. Z.High Field 27Al MAS NMR and TPD Studies of Active Sites in Ethanol Dehydration Using Thermally Treated Transitional Aluminas as Catalysts. J. Catal. 2016, 336, 85– 93, DOI: 10.1016/j.jcat.2016.01.006 [Crossref], [CAS], Google Scholar
16Kwak, J. H.; Hu, J.; Mei, D.; Yi, C.-W.; Kim, D. H.; Peden, C. H. F.; Allard, L. F.; Szanyi, J. Coordinatively Unsaturated Al3+ Centers as Binding Sites for Active Catalyst Phases of Platinum on γ-Al2O3. Science 2009, 325, 1670– 1673, DOI: 10.1126/science.1176745 [Crossref], [PubMed], [CAS], Google Scholar
17Zhang, Z.Thermally Stable Single Atom Pt/M-Al2O3 for Selective Hydrogenation and CO Oxidation. Nat. Commun. 2017, 8, 16100, DOI: 10.1038/s41467-017-01785-w [Crossref], [PubMed], [CAS], Google Scholar
18Duan, H.; You, R.; Xu, S.; Li, Z.; Qian, K.; Cao, T.; Huang, W.; Bao, X. Pentacoordinated Al3+-Stabilized Active Pd Structures on Al2O3-Coated Palladium Catalysts for Methane Combustion. Angew. Chem., Int. Ed. 2019, 58, 12043– 12048, DOI: 10.1002/anie.201904883 [Crossref], [CAS], Google Scholar
19Tang, N.; Cong, Y.; Shang, Q.; Wu, C.; Xu, G.; Wang, X. Coordinatively Unsaturated Al3+ Sites Anchored Subnanometric Ruthenium Catalyst for Hydrogenation of Aromatics. ACS Catal. 2017, 7, 5987– 5991, DOI: 10.1021/acscatal.7b01816 [ACS Full Text ACS Full Text], [CAS], Google Scholar
20Mei, D.; Kwak, J. H.; Hu, J.; Cho, S. J.; Szanyi, J.; Allard, L. F.; Peden, C. H. F. Unique Role of Anchoring Penta-Coordinated Al3+ Sites in the Sintering of γ-Al2O3-Supported Pt Catalysts. J. Phys. Chem. Lett. 2010, 1, 2688– 2691, DOI: 10.1021/jz101073p [ACS Full Text ACS Full Text], [CAS], Google Scholar
21Düvel, A.; Romanova, E.; Sharifi, M.; Freude, D.; Wark, M.; Heitjans, P.; Wilkening, M. Mechanically Induced Phase Transformation of γ-Al2O3 into α-Al2O3. Access to Structurally Disordered γ-Al2O3 with a Controllable Amount of Pentacoordinated Al Sites. J. Phys. Chem. C 2011, 115, 22770– 22780, DOI: 10.1021/jp206077r [ACS Full Text ACS Full Text], [CAS], Google Scholar
22Chen, S.; Chen, J.; Qu, T.; Xiang, K.; Zhang, Y.; Hao, P.; Peng, L.; Xie, M.; Guo, X.; Ding, W. Crown Ether Induced Assembly to γ-Al2O3 Nanosheets with Rich Pentacoordinate Al3+ Sites and High Ethanol Dehydration Activity. Appl. Surf. Sci. 2018, 457, 626– 632, DOI: 10.1016/j.apsusc.2018.06.210 [Crossref], [CAS], Google Scholar
23Yuan, Q.; Yin, A.-X.; Luo, C.; Sun, L.-D.; Zhang, Y.-W.; Duan, W.-T.; Liu, H.-C.; Yan, C.-H. Facile Synthesis for Ordered Mesoporous γ-Aluminas with High Thermal Stability. J. Am. Chem. Soc. 2008, 130, 3465– 3472, DOI: 10.1021/ja0764308 [ACS Full Text ACS Full Text], [CAS], Google Scholar
24Shi, L.; Deng, G.-M.; Li, W.-C.; Miao, S.; Wang, Q.-N.; Zhang, W.-P.; Lu, A.-H. Al2O3 Nanosheets Rich in Pentacoordinate Al3+ Ions Stabilize Pt-Sn Clusters for Propane Dehydrogenation. Angew. Chem., Int. Ed. 2015, 54, 13994– 13998, DOI: 10.1002/anie.201507119 [Crossref], [CAS], Google Scholar
25Kwak, J. H.; Hu, J.; Lukaski, A.; Kim, D. H.; Szanyi, J.; Peden, C. H. F. Role of Pentacoordinated Al3+ Ions in the High Temperature Phase Transformation of γ-Al2O3. J. Phys. Chem. C 2008, 112, 9486– 9492, DOI: 10.1021/jp802631u [ACS Full Text ACS Full Text], [CAS], Google Scholar
26Wischert, R.; Copéret, C.; Delbecq, F.; Sautet, P. Optimal Water Coverage on Alumina: A Key to Generate Lewis Acid–Base Pairs That Are Reactive Towards the C-H Bond Activation of Methane. Angew. Chem., Int. Ed. 2011, 50, 3202– 3205, DOI: 10.1002/anie.201006794 [Crossref], [CAS], Google Scholar
27Wischert, R.; Laurent, P.; Copéret, C.; Delbecq, F.; Sautet, P. γ-Alumina: The Essential and Unexpected Role of Water for the Structure, Stability, and Reactivity of “Defect” Sites. J. Am. Chem. Soc. 2012, 134, 14430– 14449, DOI: 10.1021/ja3042383 [ACS Full Text ACS Full Text], [CAS], Google Scholar
28Digne, M.; Sautet, P.; Raybaud, P.; Euzen, P.; Toulhoat, H. Use of DFT to Achieve a Rational Understanding of Acid–Basic Properties of γ-Alumina Surfaces. J. Catal. 2004, 226, 54– 68, DOI: 10.1016/j.jcat.2004.04.020 [Crossref], [CAS], Google Scholar
29Pinto, H. P.; Nieminen, R. M.; Elliott, S. D. Ab Initio Study of γ-Al2O3 Surfaces. Phys. Rev. B 2004, 70, 125402, DOI: 10.1103/PhysRevB.70.125402 [Crossref], [CAS], Google Scholar
30Khivantsev, K.; Jaegers, N. R.; Kwak, J.-H.; Szanyi, J.; Kovarik, L. Precise Identification and Characterization of Catalytically Active Sites on the Surface of γ-Alumina. Angew. Chem., Int. Ed. 2021, 60, 17522– 17530, DOI: 10.1002/anie.202102106 [Crossref], [CAS], Google Scholar
31Bonhomme, C.; Coelho, C.; Baccile, N.; Gervais, C.; Azaïs, T.; Babonneau, F. Advanced Solid State NMR Techniques for the Characterization of Sol–Gel-Derived Materials. Acc. Chem. Res. 2007, 40, 738– 746, DOI: 10.1021/ar600030j [ACS Full Text ACS Full Text], [CAS], Google Scholar
32Ashbrook, S. E.; Hodgkinson, P. Perspective: Current Advances in Solid-State NMR Spectroscopy. J. Chem. Phys. 2018, 149, 040901, DOI: 10.1063/1.5038547 [Crossref], [PubMed], [CAS], Google Scholar
33Li, S.; Lafon, O.; Wang, W.; Wang, Q.; Wang, X.; Li, Y.; Xu, J.; Deng, F. Recent Advances of Solid-State NMR Spectroscopy for Microporous Materials. Adv. Mater. 2020, 32, 2002879, DOI: 10.1002/adma.202002879 [Crossref], [CAS], Google Scholar
34O’Dell, L. A.; Savin, S. L. P.; Chadwick, A. V.; Smith, M. E. A 27Al MAS NMR Study of a Sol–Gel Produced Alumina: Identification of the NMR Parameters of the Θ-Al2O3 Transition Alumina Phase. Solid State Nucl. Magn. Reson. 2007, 31, 169– 173, DOI: 10.1016/j.ssnmr.2007.05.002 [Crossref], [PubMed], [CAS], Google Scholar
35Lee, D.; Duong, N. T.; Lafon, O.; De Paëpe, G. Primostrato Solid-State NMR Enhanced by Dynamic Nuclear Polarization: Pentacoordinated Al3+ Ions Are Only Located at the Surface of Hydrated γ-Alumina. J. Phys. Chem. C 2014, 118, 25065– 25076, DOI: 10.1021/jp508009x [ACS Full Text ACS Full Text], [CAS], Google Scholar
36Taoufik, M.; Szeto, K. C.; Merle, N.; Rosal, I. D.; Maron, L.; Trébosc, J.; Tricot, G.; Gauvin, R. M.; Delevoye, L. Heteronuclear NMR Spectroscopy as a Surface-Selective Technique: A Unique Look at the Hydroxyl Groups of γ-Alumina. Chem.─Eur. J. 2014, 20, 4038– 4046, DOI: 10.1002/chem.201304883 [Crossref], [PubMed], [CAS], Google Scholar
37Szeto, K. C.; Merle, N.; Trébosc, J.; Taoufik, M.; Gauvin, R. M.; Pourpoint, F.; Delevoye, L. Caveat on the Actual Robustness of Heteronuclear NMR Methods for Probing the Surface of γ-Alumina and Related Catalysts. J. Phys. Chem. C 2019, 123, 12919– 12927, DOI: 10.1021/acs.jpcc.9b02634 [ACS Full Text ACS Full Text], [CAS], Google Scholar
38Wischert, R.; Florian, P.; Copéret, C.; Massiot, D.; Sautet, P. Visibility of Al Surface Sites of γ-Alumina: A Combined Computational and Experimental Point of View. J. Phys. Chem. C 2014, 118, 15292– 15299, DOI: 10.1021/jp503277m [ACS Full Text ACS Full Text], [CAS], Google Scholar
39Wang, J.; Lu, A.-H.; Li, M.; Zhang, W.; Chen, Y.-S.; Tian, D.-X.; Li, W.-C. Thin Porous Alumina Sheets as Supports for Stabilizing Gold Nanoparticles. ACS Nano 2013, 7, 4902– 4910, DOI: 10.1021/nn401446p [ACS Full Text ACS Full Text], [CAS], Google Scholar
40Massiot, D.; Fayon, F.; Capron, M.; King, I.; Le Calvé, S.; Alonso, B.; Durand, J.-O.; Bujoli, B.; Gan, Z.; Hoatson, G. Modelling One- and Two-Dimensional Solid-State NMR Spectra. Magn. Reson. Chem. 2002, 40, 70– 76, DOI: 10.1002/mrc.984 [Crossref], [CAS], Google Scholar
41Shen, L.; Wang, Y.; Du, J.-H.; Chen, K.; Lin, Z.; Wen, Y.; Hung, I.; Gan, Z.; Peng, L. Probing Interactions of γ-Alumina with Water Via Multinuclear Solid-State NMR Spectroscopy. ChemCatChem. 2020, 12, 1569– 1574, DOI: 10.1002/cctc.201901838 [Crossref], [PubMed], [CAS], Google Scholar
42Wang, F.; Ma, J.; Xin, S.; Wang, Q.; Xu, J.; Zhang, C.; He, H.; Cheng Zeng, X. Resolving the Puzzle of Single-Atom Silver Dispersion on Nanosized γ-Al2O3 Surface for High Catalytic Performance. Nat. Commun. 2020, 11, 529, DOI: 10.1038/s41467-019-13937-1 [Crossref], [PubMed], [CAS], Google Scholar
43Bax, A. Structure Determination and Spectral Assignment by Pulsed Polarization Transfer Via Long-Range 1H 13C Couplings. J. Magn. Reson. 1984, 57, 314– 318, DOI: 10.1016/0022-2364(84)90133-1 [Crossref], [CAS], Google Scholar
44Amoureux, J. P.; Trebosc, J.; Wiench, J.; Pruski, M. HMQC and Refocused-Inept Experiments Involving Half-Integer Quadrupolar Nuclei in Solids. J. Magn. Reson. 2007, 184, 1– 14, DOI: 10.1016/j.jmr.2006.09.009 [Crossref], [PubMed], [CAS], Google Scholar
45Iuga, D. Double-Quantum Homonuclear Correlations of Spin I = 5/2 Nuclei. J. Magn. Reson. 2011, 208, 225– 234, DOI: 10.1016/j.jmr.2010.11.007 [Crossref], [PubMed], [CAS], Google Scholar
46Lee, D.; Takahashi, H.; Thankamony, A. S. L.; Dacquin, J.-P.; Bardet, M.; Lafon, O.; De Paëpe, G. Enhanced Solid-State NMR Correlation Spectroscopy of Quadrupolar Nuclei Using Dynamic Nuclear Polarization. J. Am. Chem. Soc. 2012, 134, 18491– 18494, DOI: 10.1021/ja307755t [ACS Full Text ACS Full Text], [CAS], Google Scholar
47Rozita, Y.; Brydson, R.; Scott, A. J. An Investigation of Commercial Gamma-Al2O3 Nanoparticles. J. Phys.: Conf. Ser. 2010, 241, 012096, DOI: 10.1088/1742-6596/241/1/012096 [Crossref], Google Scholar
48Swaddle, T. W.; Rosenqvist, J.; Yu, P.; Bylaska, E.; Phillips, B. L.; Casey, W. H. Kinetic Evidence for Five-Coordination in AlOH(Aq)2+ Ion. Science 2005, 308, 1450– 1453, DOI: 10.1126/science.1110231 [Crossref], [PubMed], [CAS], Google Scholar
49Taulelle, F.; Pruski, M.; Amoureux, J. P.; Lang, D.; Bailly, A.; Huguenard, C.; Haouas, M.; Gérardin, C.; Loiseau, T.; Férey, G. Isomerization of the Prenucleation Building Unit During Crystallization of AlPO4-CJ2: An MQMAS, CP-MQMAS, and HETCOR NMR Study. J. Am. Chem. Soc. 1999, 121, 12148– 12153, DOI: 10.1021/ja991295n [ACS Full Text ACS Full Text], [CAS], Google Scholar
50Ahrem, L.; Scholz, G.; Gutmann, T.; Calvo, B.; Buntkowsky, G.; Kemnitz, E. Direct Observation of Coordinatively Unsaturated Sites on the Surface of a Fluoride-Doped Alumina Catalyst. J. Phys. Chem. C 2017, 121, 12206– 12213, DOI: 10.1021/acs.jpcc.7b02535 [ACS Full Text ACS Full Text], [CAS], Google Scholar
51Prins, R. On the Structure of γ-Al2O3. J. Catal. 2020, 392, 336– 346, DOI: 10.1016/j.jcat.2020.10.010 [Crossref], [CAS], Google Scholar
52Slade, R. C. T.; Southern, J. C.; Thompson, I. M. 27Al Nuclear Magnetic Resonance Spectroscopy Investigation of Thermal Transformation Sequences of Alumina Hydrates. Part 1.─Gibbsite, γ-Al(OH)3. J. Mater. Chem. 1991, 1, 563– 568, DOI: 10.1039/JM9910100563 [Crossref], [CAS], Google Scholar
53Cui, J. L.Aluminum Oxide Thin Films from Aqueous Solutions: Insights from Solid-State NMR and Dielectric Response. Chem. Mater. 2018, 30, 7456– 7463, DOI: 10.1021/acs.chemmater.7b05078 [ACS Full Text ACS Full Text], [CAS], Google Scholar
54Sarou-Kanian, V.; Gleizes, A. N.; Florian, P.; Samélor, D.; Massiot, D.; Vahlas, C. Temperature-Dependent 4-, 5- and 6-Fold Coordination of Aluminum in MOCVD-Grown Amorphous Alumina Films: A Very High Field 27Al-NMR Study. J. Phys. Chem. C 2013, 117, 21965– 21971, DOI: 10.1021/jp4077504 [ACS Full Text ACS Full Text], [CAS], Google Scholar