Optimizing Aluminum Oxide Coatings for Li-ion Cathodes
Understanding the Role of Temperature and Cathode Composition on Interface and Bulk
Binghong Han1, Tadas Paulauskas2, Baris Key1, Cameron Peebles 1, Joong-Sun Park1, Robert F. Klie2,John T. Vaughey1, Fulya Dogan1*
1. Chemical Sciences and Engineering Division, Argonne National Laboratory, Argonne, Illinois 60439, United States
2. Department of Physics, University of Illinois at Chicago, 845 W Taylor Street, Chicago, IL 60607, USA
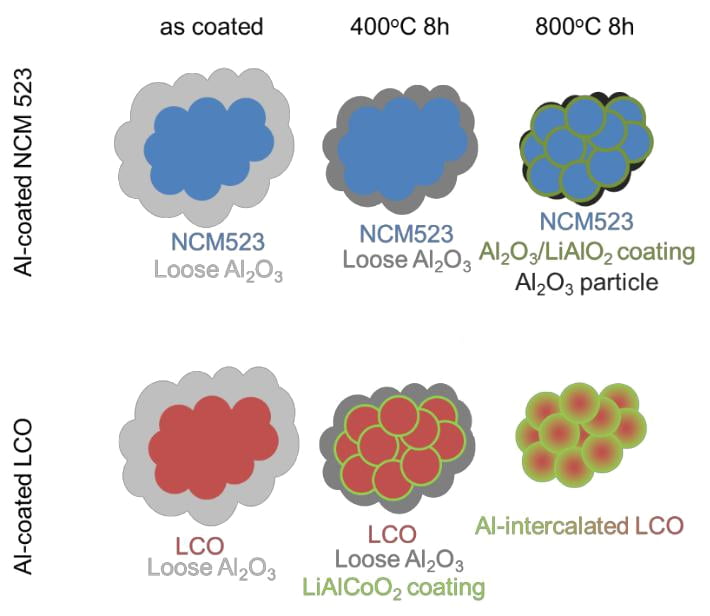
Keywords : NMC, LCO, Al2O3 coating, coating vs doping, TEM, 27Al MAS NMR
ABSTRACT
Surface coating of cathode materials with Al2O3 has been shown to be a promising method for cathode stabilization and improved cycling performance at high operating voltages. However, a detailed understanding on how coating process and cathode composition changes the chemical composition, morphology and distribution of coating within cathode interface and bulk lattice, is still missing. In this study, we use a wet-chemical method to synthesize a series of Al2O3-coated LiNi0.5Co0.2Mn0.3O2 and LiCoO2 cathodes treated under various annealing temperatures and a combination of structural characterization techniques to understand the composition, homogeneity and morphology of coating layer and the bulk cathode. Nuclear magnetic resonance and electron microscopy results reveal that the nature of the interface is highly depended on the annealing temperature and cathode composition. For Al2O3-coated LiNi0.5Co0.2Mn0.3O2, higher annealing temperature leads to more homogeneous and more closely attached coating on cathode materials, corresponding to better electrochemical performance. Lower Al2O3 coating content is found to be helpful to further improve the initial capacity and cyclability, which can greatly outperform the pristine cathode material. For Al2O3-coated LiCoO2, the incorporation of Al into the cathode lattice is observed after annealing at high temperatures, implying the transformation from “surface coatings” to “dopants”, which is not observed for LiNi0.5Co0.2Mn0.3O2. As a result, Al2O3-coated LiCoO2 annealed at higher temperature shows similar initial capacity but lower retention compared to that annealed at a lower temperature, due to the intercalation of surface alumina into the bulk layered structure forming a solid solution.
INTRODUCTION
The fast development of portable electronic devices and zero-emission or hybrid electric vehicles has raised great research interests in developing new lithium-ion batteries with higher energy density and longer life time1-2. In the last two decades, many Ni- and Mn-based layered compounds with the compositions of LiNixCoyMn1-x-yO2 (NCM) have been studied as promising cathode materials, with higher energy density, better thermal stability, and relatively low costs compared with the traditional LiCoO2 (LCO) cathode materials3-11. It is believed that adding Ni can improve the capacity and adding Mn can enhance the stability of the layered cathode materials3-4. However, when charged to a high voltage (4.6 V or higher), NCM type of materials still show instability due to increased electrode surface reactions (solid electrolyte interphase formation), electrolyte decomposition, transition metal dissolution and cathode surface transformations due to transition metal rearrangement and oxygen loss4. Previous studies on LCO has shown that the surface coating of MgO,12 SiO2,13 TiO2,14 ZrO2,15 ZnO,16 and particularly the commonly used Al2O317-19 can effectively improve the electrochemical performance and/or cyclability of LCO by suppressing the chemical and structural transitions during the electrochemical cycles17, 20. Similar coating approaches have also been applied to NCM system to decrease metal dissolution and protect electrode surface interacting with electrolyte21-26. Two commonly used surface Al2O3 coatings methods are wet-chemical process21-23 and atomic layer deposition (ALD) process24-26. Compared to ALD, the wet-chemical method is cheaper, simpler and easier to scale up, however it has less control on the coating quality and requires post heat treatment27-28.
Although previous studies have shown improved performance on Al2O3-coated NCM and LCO cathodes through a wet chemical process18-19, 21-23, a detailed understanding on the effects of sintering conditions and cathode compositions to the coating layers is still missing. Particularly, in previous cases the Al2O3-coated cathode materials were often characterized by long-range-order techniques, e.g. X-ray diffraction (XRD), and therefore little has been studied regarding to the aluminum local environment and the composition of the interface between the coating layer and bulk cathode, which are important for the surface-electrolyte interaction and hence the solid electrolyte interface (SEI) formation. The effect of sintering conditions on homogeneity, morphology and thickness of the coating layers is also critical as they can affect the overall electrochemical performance and stability. Understanding how the coating process and cathode composition affect the interfacial and bulk chemistry and hence the electrochemical performance of Ni-rich cathodes is important for the future development of surface-modified NCM cathodes. Our previous experimental study on aluminum-doped cathodes (LiNi1–y–zCoyAlzO2 (NCA) and NCM) showed Al-Mn incompatibility and favored aluminum solubility in cobalt-rich systems29. Therefore it is critical to have compositional local characterization on coated cathodes to look for possible transformation from surface coatings to a dopant.
In this study, a series of Al2O3-coated LiNi0.5Co0.2Mn0.3O2 (NCM523) and LCO are prepared through wet coating method and treated under various sintering conditions. XRD, Scanning Electron Microscopy (SEM), Transmission Electron Microscopy (TEM) and solid state Nuclear Magnetic Resonance (NMR) characterization techniques have been applied to study the evolution of surface species, possible aluminum diffusion into the lattice, changes in coating morphology and thickness, and changes on the long range order of the coated materials upon high temperature treatment. Local structural characterization data have shown the effect of cathode composition on aluminum diffusion into the lattice, in other words expected coating converting into a dopant with annealing process. These new findings can help us find out how the coating process, chemical structure, surface morphology and layer thickness of surface coating can influence the electrochemical performance of the cathode materials, which is critical to develop new design principles of next-generation cathode materials with improved stability and capacity.
EXPERIMENTAL SECTION
II.A. Material Preparation. The pristine NCM523 and LCO were commercial powders from TODA America Inc. During the coating process, the cathode powders were first added into the aqueous solution made of Al(NO3)3∙9H2O (Sigma Aldrich) and DI water. The ratios between Al and pristine cathode powders were determined to produce a final Al2O3 ratio of 2, 1, 0.5 or 0 wt% (the 0 wt% one is noted as the water-treated sample). The mixture was stirred at room temperature for 6 h. Then the temperature was raised to 80 oC and the stirring was continued overnight to evaporate the water. The left mixture was further dried in the vacuum for 4 h. The obtained as-coated powders were annealed at various temperatures (200, 400, 600 or 800 oC) for 8 h to assess the reactivity of the coating with the cathode and its effect on cathode surface and bulk structure.
II.B. Electrochemical Testing. Electrochemical tests were performed using 2032-type coin cells containing cathode electrodes prepared by two methods. The first method consisted of making a slurry from acetone and NCM523 powders (pristine and coated) that were mixed with Super P carbon (10% wt). The slurry was drop-cast on the stainless steel spacers/current collectors of the coin cell assemblies, thoroughly dried, and then assembled into cells as usual. The second method consisted of laminating Al foil current collectors with a slurry containing 82 wt% of the oxide powder, 8wt% Super P carbon, and 10 wt% polyvinylidene difluoride (PVDF) binder in N-Methyl 2-Pyrrolidone (NMP). The electrolyte was a 1.2 M solution of LiPF6 in a 3:7 mixture of ethylene carbonate (EC) and ethylmethyl carbonate (EMC). The separators used were glass fiber for the first method and Celgard-2325 for the second respectively. Lithium metal anodes were used and the cycling conditions are included in Figure captions for electrochemical testing data accordingly. After electrochemical testing, the cells were disassembled in an Ar-filled glove box. The cathodes were rinsed with anhydrous DMC to remove electrolyte and dried in Ar before further characterizations.
II.C. Structural Characterizations. Powder XRD patterns for the synthesized samples were obtained using Bruker D8 diffractometer equipped with Cu–Kα radiation source (λ = 1.5418 Å). The lattice parameters were obtained by doing Rietveld refinement on the XRD patterns using Profex software (version 3.8.0).
27Al Magic angle spinning (MAS) NMR spectra were acquired at 11.7 T, 500 MHz on Bruker Avance
spectrometer at a Larmor frequency of 130.25 MHz. A rotor-synchronized echo pulse sequence with the pulse width of 1.5 μs was used to acquire the spectra with a 2.5 mm probe at spinning speed of 30 kHz. The pulse delay times was 2 s. All spectra were collected at a constant temperature of 283 K. The spectra were referenced to 1 M Al(NO3)3at 0 ppm.
SEM images were taken using a Hitachi S-4700-II microscope in the Electron Microscopy Center of Argonne National Laboratory. The dry powders were directly sprayed onto the conductive carbon tape before imaging. Images were taken at 20 kV operating voltage.
TEM studies were performed at 200 kV on a probe-side aberration-corrected JEOL JEM ARM-200CF, equipped with Energy Dispersive Spectrometry (EDS). The images and EDS mappings were taken in the Scanning Transmission Electron Microscope (STEM) mode, using Gatan Digital Micrograph v2.01 (Gatan Inc.) and AZtecEnergy (Oxford Instruments) respectively.
III. RESULTS
III.A. Structural Characterization Results.
X-ray diffraction patterns for all Al2O3-coated NCM and LCO samples (annealed at different temperatures) conform to the R-3m symmetry of the pristine materials. No detectable additional peak formation was observed by diffraction for the presence of additional phases formed and the only detectable changes in diffraction analysis were unit cell volume changes. Figure 1 shows the unit cell volumes of pristine and 2% Al2O3-coated NCM523 and LCO heat treated under various temperatures, calculated from XRD refinement results. An example of XRD refinement and a full table of the lattice parameters can be found in Figure S1 and Table S1 in the Supporting Information (SI). For NCM523, the as-coated sample and the coated samples annealed at 200 and 400 oC do not show significant change in the unit cell volume, indicating the bulk of NCM523 is merely influenced by the coating or low-temperature annealing process. When the annealing temperature is above 400 oC, there is a minor increase in the unit cell volume, indicating a possible aluminum insertion or lithium loss from the structure at higher temperatures. For aluminum coated LCO, the same minor change in unit cell volume is seen even after annealing at 400 oC, indicating the crystal structure of LCO is easier to be influenced by alumina coating at lower temperatures compared to NCM523. The lack of significant observable difference in long range order from XRD warrants investigation of the material with a local probe technique. Therefore solid state NMR spectroscopy is used to study possible aluminum insertion, lithium loss and formation of new phases that are not detectable by XRD.
Figure 1. Volumes of unit cells of 2% Al2O3-coated NCM523 and LCO annealed at various temperatures for 8 h. The points at 25 oC represent the as-coated particles.
The effect of annealing temperature on aluminum local environment, possible aluminum diffusion into the lattice and composition coating layer are studied by 27Al MAS NMR, as shown in Figure 2. Solid state NMR is a powerful characterization tool and has been playing a unique and complimentary role as a probe for Li-ion systems, providing useful information on chemical and structural local environment changes, chemical bonding, local dynamics of bulk Li-ions and evolution of surface layers20. Although it is generally considered as a bulk technique, it has been increasingly utilized to study interfacial species (solid-electrolyte interphase and surface coating composition and morphology) and provide information on bulk versus surface species for paramagnetic battery systems20, 29. Due to the hyperfine (or Fermi-contact) interaction, which is a measure of the unpaired spin density that is transferred from paramagnetic transition metals ions to the s-orbital of the observed nuclei (through oxygen p-orbital) in the bulk, NMR peak positions are significantly shifted from that in diamagnetic surface environments. Therefore solid state NMR is a unique technique to study whether a coating stays as a surface layer acting as a coating or diffuses into the lattice and work as a dopant29. As seen in Figure 2a, with annealing temperature below 400 oC, only 6-coordinate Al peaks for surface Al2O3 and/or Al(OH)6 are observed in 27Al MAS NMR spectra of 2% Al2O3-coated NCM523. When the annealing temperature is increased to 600 and 800 oC, formation of lithium aluminate (LiAlO2) is observed with a new aluminum-27 peak emerging at ~69 ppm (Figure 2a). Formation of LiAlO2 also suggests possible lithium removal from bulk and formation of this surface lithium bearing species during the wet-chemical coating process. 27Al NMR data of pure LiAlO2 and Al2O3, as well as their physical mixture with NCM523 compared to Al2O3-coated NCM523 can be found in the Figure S2 and S3 in Supplemental Information for comparison. This observation is significant to understand the effect of a wet coating and temperature treatment on bulk and interface changes as well as their effect on electrochemical performance of coated cathode and will be discussed in more detail in later sections.
In addition to the formation of new aluminum environments with increasing annealing temperature on Al2O3-coated NCM523, significant changes are observed for spinning sideband pattern and envelope, as shown in Figure 2b. The formation of spinning sidebands are partially due to electron-dipolar interaction and contains information on the interaction between a surface species (nucleus under observation) and the bulk active material with paramagnetic metal centers. The dipolar interaction has a (1/r)3 dependency, where r is the distance between the two dipoles involved. Large dipolar interaction leads to a broad NMR spectra lineshape and a broad spinning sideband envelope, which corresponds to a small distance between the nucleus under observation and paramagnetic bulk30-31. Therefore the changes in spinning sideband pattern can provide information on the thickness and distribution of the surface species. On Al2O3-coated NCM523, with increasing annealing temperature, the sideband envelope gets broader and the intensity of sidebands for aluminum oxide peaks increases due to increased proximity of surface coating with paramagnetic bulk, which is likely caused by the formation of a denser, more closely attached and more uniform Al2O3 coating. These lineshapes observed are wider than the spinning sideband manifold observed for Al2O3 alone and can only be seen with strong interaction with paramagnetic centers distributed around aluminum nuclei ( as shown with spectra comparison in Figure S3).
Figure 2. (a) The near-0-ppm region of 27Al MAS NMR results of as-coated and annealed NCM523 (LiNi0.5Co0.2Mn0.3O2) samples. (b) The whole spectra of 27Al MAS NMR results of as-coated and annealed NCM523. (c) The near-0-ppm region of 27Al MAS NMR results of as-coated and annealed LCO (LiCoO2) samples, compared to LiAlCoO2 solid solution.
The effect of annealing temperature on aluminum local environments is also studied for Al2O3-coated LCO samples by solid state NMR. Figure 2c shows 27Al MAS NMR spectra comparison of as coated LCO, coated sample annealed at 400 and 800 oC and aluminum-doped lithium cobalt oxide. The as-coated sample shows a broad peak at ~ 0 ppm for six- coordinate aluminum oxide. Different from the Al2O3-coated NCM523 prepared with the coating process, Al2O3-coated LCO shows the formation of new aluminum environments with annealing temperature of 400 oC for 8 h. As the temperature increased to 800 oC, formation of at least 6 different aluminum peaks are observed from 25 ppm to 60 ppm, each separated ~ 7 ppm apart (Figure 2c). These peak positions and increment values are consistent with the previous 27Al MAS NMR studies on LiAlxCo1-xO2 solid solutions32 and the observed peaks can be assigned as octahedral aluminum environments with varying cobalt neighbors (Figure 2c). These peak observations and overlaps with our synthesized LiAlxCo1-xO2 sample prove that Al2O3-coated LCO gradually forms Al-doped LCO with increasing annealing temperature, implying the Al2O3 coating layer is diffusing into the bulk and forming a solution of LiAlCoO2. Comparing to the Al2O3-coated NCM523 samples, we can find that the Al diffusion from the surface coating layer to the bulk is much easier in LCO, which will be discussed in the later section.
The effect of annealing temperature and cathode composition on coating-cathode interface morphology and thickness is further studied by electron microscopy (Figures 3, 4 and 5). Figure 3 shows SEM images of pristine and Al2O3-coated NCM523 samples (as-coated and annealed at varying temperatures). The pristine sample has primary particles of 0.5 to 1.5 µm with smooth surfaces (Figure 3a) and secondary particles around 10 µm (image not shown). The as-coated material has a loose surface layer covering all secondary particle (Figure 3b). As the material is heated to 200 and 400 oC the loose surface layer gets denser and more closely attached to the surface, but the morphology change is very limited (Figure 3c and 3d). After annealing at 600 oC, the surface Al2O3 layer clearly transforms from loose coating to a more uniform layer attached more closely to the bulk NCM523 revealing primary particles again, as shown in Figure 3e. With heating the coated sample to 800 oC, the coating layer becomes more like a hard shell uniformly covering the bulk NCM primary particles (Figure 3f). There is no significant change observed in primary particle size with high temperature treatment. For both 600 oC and 800 oC annealed samples, some small particles can be observed decorated on the surface, which is likely due to excess Al2O3 segregated under high temperatures.
Figure 3. SEM images of (a) pristine NCM523, (b) as-coated NCM523 and (c)-(f) Al2O3-coated NCM523 annealed at various temperatures for 8 h.
To further study the morphology and thickness of the surface coating layer, low-angle annular dark field (LAADF) TEM images and EDS mapping are employed and the results are shown in Figure 4 and Figure S4, respectively. The TEM images of as-coated and 200 or 400oC-annealed NCM523 demonstrate a loose amorphous covering on top of the bulk NCM523 particle with the thickness of ~20, ~15 and ~10 nm, respectively. The identity and thickness of such Al-rich surface layer can be confirmed using EDS mapping technique under STEM mode, as shown in Figures S4b-S4d, where Al-rich layers outside the bulk particle with a thickness from ~20 to ~10 nm can be observed. With increasing the annealing temperature to 600 and 800 oC, the surface Al2O3 coating forms very thin layers closely attached to the bulk NCM523 particle (Figures 4e and 4f). These thin coating layers can be identified by comparing the bright-field (BF) image to the LAADF image taken at the same time, as shown in Figures S5e and S5f, where the different contrasts in the surface rims between the corresponding BF and LAADF images come from the higher atomic-number sensitivity of the annular dark field mode compared to the BF mode. The brighter outer rims in LAADF images in Figures S5e and S5f represent the lighter-element-rich (i.e. Al-rich) coating layers, which is only ~3 nm and ~2 nm for samples annealed at 600 oC and 800 oC, representatively. Such coating is too thin and therefore cannot be separated within the spatial resolution of EDS mapping (Figure S4e and S4f). In addition, a semi-amorphous interface layer can be observed for 600 oC annealed sample (pointed in the Figure S6a), which might be the misaligned interfacial layer between the bulk NCM523 and the surface Al-coating. On the contrary, for 800 oC annealed sample, continuous crystal fringes can be observed from the bulk to the surface (Figure S6b), implying that the coating layer is better aligned with the bulk layered structure after higher temperature annealing. These interfacial differences can influence the electrochemical performance and surface composition and morphology effects on electrochemistry will be discussed in the following section. On some selected particles, high angle annular dark field images and EDS mapping show some non-uniform Al- and O-rich regions for coated NCM523 samples annealed at 600 oC and 800 oC (Figure S7). These can represent excess segregated Al2O3 and/or LiAlO2 small particles, which are also observed in SEM images shown in Figures 3e and 3f.
Figure 4. Low-angle annular dark field TEM images of (a) pristine NCM523, (b) as-coated NCM523 and (c)-(f) Al2O3-coated NCM523 annealed at various temperatures for 8 h. The green dash lines shown in panels (b)-(f) represents the rough interface between the bulk and the surface coating layer, which can be confirmed by comparing the dark-field images to the corresponding bright-field images in Figure S5.
In the case of Al2O3-coated LCO, due to the feasibility of aluminum into the bulk cathode after annealing, the morphological change shows a different trend from that of coated NCM523. Figure 5 shows SEM images for pristine, as coated LCO and coated samples annealed at 400 and 800 oC. Pristine primary particle sizes are in the range of 1-3 µm whereas secondary particle sizes are around 10 µm. As seen in Figure 5b the as-coated LCO looks similar to that of as-coated NCM523 in Figure 3b with loose surface coating layers covering the secondary particle. With annealing the sample at 400 oC for 8 h, the coating gets more uniformly covering the primary particles separately as more similar to coated NCM523 primary particles annealed at 800 oC. When the annealing temperature is increased to 800 oC the coated particle surface becomes more pristine-like and no surface layer can be identified from the SEM images, which is likely the result of the total intercalation of Al into the bulk as proven by the 27Al MAS NMR results.
Figure 5. SEM images of (a) pristine LCO, (b) as-coated LCO and (c)-(d) Al2O3-coated LCO annealed at various temperatures for 8 h.
III.B. Electrochemical Test Results.
Effect of Annealing Temperature: The effect of annealing temperature on electrochemical performance of 2% Al2O3 coated NCM523 are studied on loose powder half cells. Figure 6 shows the cycling performance of pristine, and coated NCM523 annealed at varying temperatures in the 3-4.5V voltage range at a rate of C/9. Significant differences are observed in initial capacities and cycling stability for pristine and coated samples with changing annealing temperatures. As seen with the discharge capacity plot, all the coated samples show lightly low initial capacities in comparison to uncoated cathode and the Al2O3-coated NCM523 cathodes annealed at higher temperatures demonstrate higher initial capacity and better cyclability compared to the low-temperature samples. These preliminary results on electrochemical performance and both bulk and surface characterization results suggest that coated samples prepared with high temperature annealing (600 and higher) show better cycling performance, more uniform and thinner coating layer covering both primary and secondary particles. Therefore further coating work on detailed electrochemical testing on effect of coating content and coating layer thickness has been focused on samples annealed at 800 oC.
The fact that initial capacities of all Al2O3-coated NCM523 electrodes are lower than that of pristine NCM523, could be attributed to the existence of thick coating layer which may increase the resistance for Li transportation through the surface. It has to be noted that the initial coating study has been performed with relative high coating content (2% by weight) to improve the structural characterization results and the effect of low coating levels on electrochemical performance will be discussed in the following section. One other possible explanation for lower initial capacities for coated samples might be possible lithium loss from bulk and formation of surface lithium bearing species after a wet coating process. This surface lithium segregation can be confirmed by comparison of normalized 7Li MAS NMR spectra for pristine, as coated and coated and annealed NCM523 samples as shown in Figure S10. The as-coated sample has sharp and high intensity lithium-7 peak at around 0 ppm for surface lithium bearing species in comparison to pristine
untreated NCM523. This shows that the aqueous coating process draws out small amount of surface lithium ions (with possible proton exchange) therefore the effect of water treatment (without any coating) needs to be studied to see its effect of electrochemical performance. As the annealing temperature increases the peak gets broader and the area integration of the peaks show decrease in surface lithium environments suggesting some of the surface lithium migrates back into the lattice with heat treatment. The broadening observed is due formation of various lithium environments with temperature increase (such as LiAlO2 for 800 oC).
For Al2O3-coated LCO, coated LCO cathodes annealed at 400 and 800 oC also show lower initial discharge capacity compared to the pristine LCO sample, but they show better cycle stability after 20 cycles (Figure 7). The lower initial capacities of Al2O3-coated LCO could be explained by the presence of electrochemically inactive Al3+ ions and the higher lithium intercalation potentials of LiCo1-xAlxO2 compounds compared to LiCoO2. Different from the Al2O3-coated NCM523, for Al2O3-coated LCO, annealing at 400 oC shows slight improvement in electrochemical performance compared to sample annealed at 800 oC. This could be due to the intercalation of surface Al into LCO starting at low temperature, unlike on NCM523 where the surface Al coating cannot intercalate into the bulk and will only start to form the dense coating at high temperatures. In addition, since aluminum intercalation is not complete (as in coated sample annealed at 800 oC) and there is still surface Al2O3 species, the stabilization effect of the surface Al coating is more pronounced. As shown in Figure 7 and Table S2, although the initial capacity of Al2O3-coated LCO (~180 mAh/g) is lower than that of the pristine LCO (~200 mAh/g), after ~20 cycles, the discharge capacity of the Al2O3-coated LCO surpasses that of pristine LCO due to the better cyclability. After 50 cycles at the rate of C/9, the relative capacity losses of the Al2O3-coated LCO are less than 20% (see Table S2), much lower than that of pristine LCO (36.4%). In comparison, due to the good cyclability of pristine NCM523, the performance of 2%Al2O3-coated NCM523 can hardly surpass its pristine counterpart even for the best coated one annealed at 800 oC. To obtain improvement both in capacity and cycling stability, a thinner coating layer with same interfacial composition (annealed at 800 oC) is also studied and will be discussed in the next section.
Figure 6. Evolution of discharge capacity for pristine NCM523 and 2%Al2O3-coated NCM523 annealed at various temperatures for 8 h as the cathode materials. The cells were cycled between 3 and 4.5 V at the rate of C/9. For each sample, two coin cells were made to ensure the repeatability of the results.
Figure 7. Evolution of discharge capacity for pristine LCO and 2%Al2O3-coated LCO annealed at 400 or 800 oC for 8 h as the cathode materials. The cells were cycled between 3 and 4.5 V at the rate of C/9.
The effect of cycling on aluminum local environment changes is studied by 27Al solid state NMR on cycled Al2O3 coated NCM523 and LCO annealed at 800 oC (Figure S8). For NCM523 where aluminum is found as Al2O3 and LiAlO2 coatings, no major change is observed for LiAlO2 phase intensity and local order. The 27Al peak for Al2O3 environment gets broader as a sign of site distortion and distribution of different aluminum environments. However these are still mostly oxide environments as 19F MAS NMR on the cycled sample does not show any formation of AlF or AlOF species (Figure S9). The 27Al MAS NMR of cathode harvested from cycled LCO cell (coated and annealed at 800 oC) is compared with pristine coated LCO in Figure S8b. It is clearly seen that the aluminum local ordering is lost with cycling and completely disordered aluminum phase is formed. This significant change is also reveals that aluminums are in the structure and not on the surface as an oxide coating.
The Role of Thinner Coating on Improved Electrochemistry and Effect of Water Treatment on Initial Capacity Loss: As briefly discussed in the previous section, the initial capacity differences observed for the coated can be due to the formation of surface species (as a result of water treatment) and/or the presence of a thick coating layer. To study the effect of these two concepts on capacity loss, new batches of Al2O3-coated NCM523 are prepared with lower coating loadings (0.5% and 1% by weight, all annealed at 800 oC for 8h); and a water-treatment control group is prepared by immersing NCM523 into the water, stirring for the same duration as the coated samples (without adding Al salt) and then annealing at 800 oC for 8h. Figure 8 and Table S3 demonstrate the electrochemical performance of pristine, water-treated, and 0.5%/1.0%/2.0% Al2O3-coated NCM523 particles, tested by the accelerate protocol (i.e. first 4 cycles at a slow rate of C/10 for SEI formation, then 80 cycles at a fast rate of C/3 for cyclability test). When decreasing the coating content from 2% to 1% and 0.5%, the initial capacity at C/10 increases from 168.5 to 176.4 and 179.4 mA/g (Table S3), implying the thinner coating can reduce the initial capacity loss caused by the Li conduction resistance from the surface coating. This is consistent to the previous study showing that when Li passes through the Al2O3 coating, it will first lithiate the surface Al2O3 layer to form a Li-conductive LixAl2O3 phase, and then overflow through the lithiated surface coating layer, which can cause the extra capacitance loss 33. In comparison to the pristine sample, the water treatment decreases the initial capacity from 188.4 to 182.6 mAh/g, proving lithium loss from the bulk during the wet-coating process also contributes to the initial capacity loss. However, this initial capacity loss is compensated with the use of thinner, well uniform coating covering both primary and secondary NCM523 particles as with the electrochemical performance stability for the cells prepared with 0.5 and 1 % Al2O3 coating. The 0.5%Al2O3-coated NCM523 shows a much smaller capacity loss (5.6%) after 80 cycles at C/3 compared to both 2%Al2O3-coated NCM523 (22.0%) and pristine NCM523 (37.6%). The discharge capacity of 0.5%Al2O3-coated NCM523 surpasses that of pristine NCM523 after ~25 cycles at C/3, implying that a reasonable thin coating is an effective way to improve the electrochemical performance of NCM cathode materials. In our test, the water treatment does not show clear influence to the cyclability, implying the influence of water treatment might be confined to the initial Li leaching and has less continuous effects to long-term performance.
Figure 8. Evolution of discharge capacity for pristine, water treated and Al2O3-coated NCM523 with various coating loadings and annealed at 800 oC for 8 h. The half- cells were cycled between 3 and 4.5 V, first at the rate of C/10 for 4 cycles (formation cycles), then at C/3 for 80 cycles.
DISCUSSIONS
IV.A. Evolution of Surface Morphology and Composition with Varying Annealing Temperature:
Al2O3-Coated NCM523. With the combination of all the results from XRD, NMR and electron microscopy studies discussed in previous sections, here we propose a scheme for the evolution of surface Al2O3 coating layer as a function of sintering temperature on NCM523, as shown in Figure 9a. The initial coating step without any temperature treatment first forms a loose and non-uniformly distributed amorphous Al2O3 and/or Al(OH)3) layer covering just the surface of secondary particles with a thickness of ~20 nm, as shown by the SEM and TEM images in Figures 3b and 4b. With annealing at lower temperatures (200 and 400 oC), the SEM and TEM images (Figures 3c, 3d, 4c and 4d) reveal that the loose oxide layer gets thinner (~10 nm) and denser covering over the secondary particles but interacting more with the bulk cathode particles. 27Al MAS NMR characterization shows the only presence of octahedral aluminum oxide as coating composition and lithium -7 solid state NMR shows the formation of surface lithium bearing species such as Li2CO3, LiOH, LiOH∙H2O and Li2O. Therefore the wet-coating process draws out a small amount of surface lithium at expense of bulk lithium. Despite these surface formation and lithium loss, the XRD results indicate that the bulk lattice is merely influenced by the coating process. When the annealing temperature is increased to 600 and 800 oC, surface lithium reacts with alumina forming a g-LiAlO2 phase, where aluminums are at tethrahedral positions. At these temperatures, the interface and coating layer become a mixture of LiAlO2 and alumina. Magnetic coupling of transition metals in NMC composition inhibits aluminum diffusion into the transition metal layer (formation of lattice aluminum) at these annealing temperatures as no paramagnetic aluminum-27 NMR shift is observed for the high temperature annealed samples. These findings are in agreement with earlier calculations from Ceder group and our experimental findings on aluminum “doped” NMC cathodes29, 34. Since the surface Al cannot diffuse into the bulk even under the high annealing temperatures, the expansion of unit cell observed from XRD results in Figure 1 on 2% Al2O3-coated NCM523 is likely caused by the Li loss from the structure rather than the Al insertion. When annealed at lower temperatures, the surface Al2O3 coating will not react with the bulk NCM523 to form new LiAlO2 phase, and therefore no lattice expansion was observed in XRD after annealed at lower temperatures. The high temperature process also forms thinner and more uniformly distributed crystal layer on the surface cathode particles with a thickness of ~3 nm (see Figures 4e and S5e), and the unit cell volume of the layered oxides increases (see Figure 1). The observation of broader spinning sideband envelope of 27Al MAS NMR signal at 600 and 800 oC shows increased intimacy of surface layer with paramagnetic bulk as and hence reduced distance between surface Al coating and the bulk NCM particle. This observation points out that the coating layer is now covering primary particles and possibly accumulating at the grain boundaries. It has to be noted that, the morphological uniformity of this interfacial layer (which is a mixture of both LiAlO2 and Al2O3) is yet to be proven with further experiments by identifying the precise distribution of this dense surface layer.
All the findings on aluminum based surface coating evolution on NCM523 cathode with changing annealing temperature are summarized within Figure 9a. This picture of surface coating evolution built on structural characterizations in combination with our electrochemical characterization data can help us to understand how the annealing temperature could influence the electrochemical performance of Al2O3-Coated NCM523. The enhanced coating layer formed at high temperatures is observed to protect the bulk NCM523 much better from the structural change during electrochemical cycles, and therefore the Al2O3-coated NCM523 annealed at higher temperatures (600 and 800 oC) demonstrate better cyclability compared to that annealed at lower temperatures (200 and 400 oC). When we gradually increase the annealing temperature, much denser and more uniform aluminum based coating layer is formed, which is more closely attached to the bulk, covering even the primary particles. At this stage the composition of the interface is a mixture of LiAlO2 and Al2O3. Presence of LiAlO2 (being a better lithium ion conductor relative to Al2O3) could help to reduce the resistance of Li transportation during the charging/discharging process. As the result, the samples heated at higher temperatures show higher initial discharge capacities (see Figure 6), and under the same coating conditions when the coating content is reduced (and the coating layer gets even thinner and more uniform) both the initial capacity and capacity retention in subsequent cycles improve (see Figure 8).
IV.B. From Coating to Dopant: Temperature Effect on Al2O3-Coated LCO. Compared to the high temperature annealed Al2O3-coated NCM523 samples, where no aluminum diffusion into the lattice is observed, local structural characterization studies revealed that Al diffusion from the surface coating layer to the bulk is much easier for lithium cobalt oxide composition. 27Al MAS NMR data shows that the LiAlCoO2 solid solution phase starts to form at only 400 oC (see Figure 2c), and at 800 oC the surface aluminum completely intercalates into the lattice. The transition of aluminum from “surface coating” to “bulk dopant” after high-temperature annealing (up to 800 oC) can only be observed for LCO but not for NCM523 composition, emphasizing the bulk chemistry has big influence on the evolution and intercalation of surface Al-based coating layers. This observation is consistent with the previous simulation studies stating that the existence of Mn in the layered oxides would increase the energy penalty and prevent the intercalation of Al into the lattice of layered oxides,34 while the existence of M = Co, Ni or Cr would not hinder the formation of Li(Al,M)O2 solid solution. Our high temperature annealed coating studies on different NCM compositions with lower manganese content (NCM 811 and NCM 622) have also shown limited aluminum diffusion into the lattice forming a combination of coated/ doped cathode with hindered electrochemical performance in comparison to the coated NCM523. These results will be subject of another article.
The intercalation of Al into the bulk of LCO after annealing leads to a different temperature effect on electrochemical performance compared to the Al2O3-coated NCM523. For Al2O3-coated LCO, the Al starts to intercalate into LCO even at 400 oC and therefore the resistance of Li transportation from the coating layer had already reduced a lot for the sample annealed at 400 oC. As a result, the difference of initial capacities between 400 oC and 800 oC annealed Al2O3-coated LCO are smaller than that of NCM523, as shown in Figure 7. Meanwhile, the Al2O3-coated LCO annealed at 400 oC has a better cyclability than that annealed at 800 oC, which is opposite to the trend in Al2O3-coated NCM523. The total intercalation of Al into the bulk and the disappearing of protecting surface Al2O3 coating are likely the reasons for the poor cyclability of 800 oC annealed Al2O3-coated LCO.
Figure 9. Schemes of the Al2O3 coating layer evolution on (a) NCM523 and (b) LCO, under different annealing temperatures.
CONCLUSIONS
In this study, we show that the evolution of surface alumina coating layer after heat treatment is highly dependent on the compositions of bulk cathodes. Based on XRD, NMR and electron microscopy results, two different models are proposed for evolution of the interface of alumina coated NCM523 and LCO with annealing at different conditions. The proposed models can be used to understand the influence of annealing temperature on coating morphology, thickness and hence to the electrochemical performance. For NCM523, after annealing at higher temperatures, the Al2O3 coating layers became denser, more closely attached and more uniformly distributed, and a new LiAlO2 phase is formed. However, no solid solution forms by Al intercalation into the bulk even after annealing Al2O3-coated NCM523 at high temperatures, proving Al intercalation is not preferred in the presence of both manganese and nickel. However, it has to be noted that this observation is only for NCM523 composition and similar studies on different NCM compositions (e.g. 442, 622 and 811) is ongoing and will be the subject of a follow-up paper. In contrast to NCM523 cathodes, for Al2O3-coated LCO, a transition of Al2O3 from surface coating to a dopant can be observed even at low annealing temperatures, and the alumina can completely diffuse into the lattice at high temperatures. As a result, the Al2O3-coated LCO samples annealed at higher temperatures shows similar initial capacity but worse cyclability during the charge/discharge cycles compared to that annealed at lower temperatures.
The electrochemical characterizations show that the Al2O3-coated NCM523 samples annealed at higher temperatures have higher initial capacities and better cyclabilities during the charge/discharge cycles compared to that annealed at lower temperatures, due to the thinner, denser and more uniform coating that improves the Li transportation and bulk chemistry protection. However even after annealing at 800 oC, with 2% Al2O3 coating content and ~2 nm thickness of coating layer, the initial capacities of Al2O3-coated NCM523 are still lower than the pristine electrodes, due to the Li transportation barrier through the thick coating layer and the Li leaching during water treatment. Our studies on the role of lower coating content and hence thinner coating (after annealing at 800 oC) have shown that the discharge capacity over 80 cycles can be greatly improved and surpassed that of the pristine electrode when the coating content is down to 0.5 %. This proves that a combination of optimized coating content and annealing temperature can provide an effective interfacial layer to improve the battery performance. The results provide significant understanding in choosing optimum coating conditions for specific cathode compositions and therefore will greatly impact development of better cathode materials for lithium ion battery applications.
ASSOCIATED CONTENT
Supporting Information
The Supporting Information is available free of charge on the ACS Publications website at:
Additional electrochemical results, NMR spectra and lattice parameter information.
Author Information
Corresponding Author
*Email: fdogan@anl.gov
Notes
The authors declare no competing financial interest.
ACKNOWLEDGEMENT
Support from the Vehicle Technologies Program, Hybrid and Electric Systems, in particular, David Howell, Tien Duong, and Peter Faguy, at the U.S. Department of Energy, Office of Energy Efficiency and Renewable Energy are gratefully acknowledged. The submitted manuscript has been created by UChicago Argonne, LLC, Operator of Argonne National Laboratory (“Argonne”). Argonne, a U.S. Department of Energy Office of Science laboratory, is operated under Contract No. DE-AC02-06CH11357. This work used SEM from the Electron Microscopy Center at Argonne, which is supported by the U. S. Department of Energy, Office of Science, Office of Basic Energy Sciences, also under Contract No. DE-AC02-06CH11357. Dr. Jason Croy is acknowledged for valuable discussions. T.P and R.F.K acknowledges funding from Joint Center for Energy Storage Research (JCESR), Energy Innovation Hub funded by the US Department of Energy, Office of Science, Basic Energy Sciences.
REFERENCES
Tarascon, J. M.; Armand, M. Issues and Challenges Facing Rechargeable Lithium Batteries. Nature2001,
414 (6861), 359-367.
Weinstock, I. B. Recent Advances in the Us Department of Energy’s Energy Storage Technology Research and Development Programs for Hybrid Electric and Electric Vehicles. Power Sources2002, 110
(2), 471-474.
Shaju, K. M.; Subba Rao, G. V.; Chowdari, B. V. R. Performance of Layered Li(Ni1/3Co1/3Mn1/3)O2as Cathode for Li-Ion Batteries. Acta 2002, 48 (2), 145-151.
Jung, S.-K.; Gwon, H.; Hong, J.; Park, K.-Y.; Seo, D.-H.; Kim, H.; Hyun, J.; Yang, W.; Kang, K. Understanding the Degradation Mechanisms of LiNi5Co0.2Mn0.3O2Cathode Material in Lithium Ion Batteries. Adv. Energy Mater. 2014, 4 (1), n/a-n/a.
Jouanneau, S.; Eberman, K. W.; Krause, L. J.; Dahn, J. R. Synthesis, Characterization, and Electrochemical Behavior of Improved Li[NixCo1−2xMnx]O2(0.1<X<0.5 ) Electrochem. Soc. 2003, 150 (12), A1637-A1642.
Lu, Z.; MacNeil, D. D.; Dahn, J. R. Layered Li[NixCo1−2xMnx]O2Cathode Materials for Lithium-Ion Batteries. Solid-State Lett. 2001, 4 (12), A200-A203.
Yabuuchi, N.; Ohzuku, T. Novel Lithium Insertion Material of LiCo1/3Ni1/3Mn1/3O2for Advanced Lithium-Ion Batteries. Power Sources 2003, 119–121, 171-174.
Ohzuku, T.; Makimura, Y. Layered Lithium Insertion Material of LiCo1/3Ni1/3Mn1/3O2for Lithium-Ion Batteries. Lett. 2001, 30 (7), 642-643.
Liu, Z.; Yu, A.; Lee, J. Y. Synthesis and Characterization of LiNi1−X−YCoxMnyO2as the Cathode Materials of Secondary Lithium Batteries. Power Sources 1999, 81–82, 416-419.
Tran, N.; Croguennec, L.; Jordy, C.; Biensan, P.; Delmas, C. Influence of the Synthesis Route on the Electrochemical Properties of LiNi425Mn0.425Co0.15O2. Solid State Ionics2005, 176 (17–18), 1539-1547.
Ma, M.; Chernova, N. A.; Toby, B. H.; Zavalij, P. Y.; Whittingham, M. S. Structural and Electrochemical Behavior of LiMn4Ni0.4Co0.2O2. J. Power Sources2007, 165 (2), 517-534.
Wang, Z.; Wu, C.; Liu, L.; Wu, F.; Chen, L.; Huang, X. Electrochemical Evaluation and Structural Characterization of Commercial LiCoO2Surfaces Modified with Mgo for Lithium-Ion Batteries. Electrochem. Soc. 2002, 149 (4), A466-A471.
Fey, G. T.-K.; Yang, H.-Z.; Prem Kumar, T.; Naik, S. P.; Chiang, A. S. T.; Lee, D.-C.; Lin, J.-R. A Simple Mechano-Thermal Coating Process for Improved Lithium Battery Cathode Materials. Power Sources2004, 132 (1–2), 172-180.
Zhang, Z.; Gong, Z.; Yang, Y. Electrochemical Performance and Surface Properties of Bare and TiO2-Coated Cathode Materials in Lithium-Ion Batteries. Phys. Chem. B2004, 108 (45), 17546-17552.
Miyashiro, H.; Yamanaka, A.; Tabuchi, M.; Seki, S.; Nakayama, M.; Ohno, Y.; Kobayashi, Y.; Mita, Y.; Usami, A.; Wakihara, M. Improvement of Degradation at Elevated Temperature and at High State-of-Charge Storage by ZrO2Coating on LiCoO2. Electrochem. Soc. 2006, 153 (2), A348-A353.
Chang, W.; Choi, J.-W.; Im, J.-C.; Lee, J. K. Effects of Zno Coating on Electrochemical Performance and Thermal Stability of LiCoO2as Cathode Material for Lithium-Ion Batteries. Power Sources 2010, 195 (1), 320-326.
Cho, J.; Kim, Y. J.; Park, B. Novel LiCoO2Cathode Material with Al2O3 Coating for a Li Ion Cell. Mater. 2000, 12 (12), 3788-3791.
Liu, L.; Wang, Z.; Li, H.; Chen, L.; Huang, X. Al2O3-Coated LiCoO2as Cathode Material for Lithium Ion Batteries. Solid State Ionics 2002, 152–153, 341-346.
Kannan, A. M.; Rabenberg, L.; Manthiram, A. High Capacity Surface-Modified LiCoO2Cathodes for Lithium-Ion Batteries. Solid-State Lett. 2003, 6 (1), A16-A18.
Fey, G. T. K.; Kao, H. M.; Muralidharan, P.; Kumar, T. P.; Cho, Y. D. Electrochemical and Solid-State Nmr Studies on LiCoO2Coated with Al2O3 Derived from Carboxylate-Alumoxane. Power Sources 2006, 163 (1), 135-143.
Cao, K.; Wang, L.; Li, L.; Liang, G. Effects of Al2O3Coating on the Structural and Electrochemical Performance of LiNi5Co0.2Mn0.3O2 Cathode Material. Appl. Mech. Mater. 2013, 320, 235-240.
Liao, J.-Y.; Manthiram, A. Surface-Modified Concentration-Gradient Ni-Rich Layered Oxide Cathodes for High-Energy Lithium-Ion Batteries. Power Sources2015, 282, 429-436.
Myung, S.-T.; Izumi, K.; Komaba, S.; Sun, Y.-K.; Yashiro, H.; Kumagai, N. Role of Alumina Coating on
Li−Ni−Co−Mn−O Particles as Positive Electrode Material for Lithium-Ion Batteries. Chem. Mater. 2005, 17 (14), 3695-3704.
Shi, Y.; Zhang, M.; Qian, D.; Meng, Y. S. Ultrathin Al2O3Coatings for Improved Cycling Performance and Thermal Stability of LiNi5Co0.2Mn0.3O2 Cathode Material. Electrochim. Acta 2016, 203, 154-161.
George, S. M. Atomic Layer Deposition: An Overview. Rev.2010, 110 (1), 111-131.
Riley, L. A.; Van Atta, S.; Cavanagh, A. S.; Yan, Y.; George, S. M.; Liu, P.; Dillon, A. C.; Lee, S.-H. Electrochemical Effects of Ald Surface Modification on Combustion Synthesized LiNi1/3Mn1/3Co1/3O2as a Layered-Cathode Material. Power Sources 2011, 196 (6), 3317-3324.
Scott, I. D.; Jung, Y. S.; Cavanagh, A. S.; Yan, Y.; Dillon, A. C.; George, S. M.; Lee, S.-H. Ultrathin Coatings on Nano-LiCoO2for Li-Ion Vehicular Applications. Nano Lett. 2011, 11 (2), 414-418.
Jung, Y. S.; Cavanagh, A. S.; Riley, L. A.; Kang, S.-H.; Dillon, A. C.; Groner, M. D.; George, S. M.; Lee, S.-H. Ultrathin Direct Atomic Layer Deposition on Composite Electrodes for Highly Durable and Safe Li-Ion Batteries. Mater.2010, 22 (19), 2172-2176.
Dogan, F.; Vaughey, J. T.; Iddir, H.; Key, B. Direct Observation of Lattice Aluminum Environments in Li Ion Cathodes LiNi1–Y–ZCoyAlzO2and Al-Doped LiNixMnyCozO2 Via 27Al MAS NMR Spectroscopy. ACS Appl. Interfaces 2016, 8 (26), 16708-16717.
Dupré, N.; Martin, J.-F.; Guyomard, D.; Yamada, A.; Kanno, R. Characterization of Interphases Appearing on LiNi5Mn0.5O2Using 7Li MAS NMR. J. Power Sources 2009, 189 (1), 557-560.
Dupre, N.; Martin, J.-F.; Guyomard, D.; Yamada, A.; Kanno, R. Detection of Surface Layers Using 7Li MAS NMR. Mater. Chem.2008, 18 (36), 4266-4273.
Gaudin, E.; Taulelle, F.; Stoyanova, R.; Zhecheva, E.; Alcántara, R.; Lavela, P.; Tirado, J. L. Cobalt(Iii) Effect on 27Al NMR Chemical Shifts in LiAlxCo1-XO2. Phys. Chem. B2001, 105 (34), 8081-8087.
Jung, S. C.; Han, Y.-K. How Do Li Atoms Pass through the Al2O3Coating Layer During Lithiation in Li-Ion Batteries? Phys. Chem. Lett. 2013, 4 (16), 2681-2685.
Buta, S.; Morgan, D.; Van der Ven, A.; Aydinol, M. K.; Ceder, G. Phase Separation Tendencies of Aluminum‐Doped Transition‐Metal Oxides ( LiAl1−XMXO2 ) in the Α ‐NaFeO2 Crystal Structure. Electrochem. Soc. 1999, 146 (12), 4335-4338.
TABLE OF CONTENTS/ABSTRACT GRAPHIC